Pushing your limits in ultra marathons demands more than just grit; it requires smart training grounded in science. Ever wonder how your body actually gets stronger between those brutal training runs? Or why sometimes more training leads to worse performance?
The answer lies in a core concept of exercise physiology: supercompensation. It’s the biological magic behind training adaptation – the body’s way of recovering beyond its previous state after stress.
But harnessing this power isn’t always straightforward. Misunderstanding the delicate balance between stress and recovery can lead to plateaus, burnout, or injury – common pitfalls for dedicated ultra runners.
This comprehensive guide provides the supercompensation principle explained
in depth, moving beyond simple definitions to explore the underlying science and practical applications.
We’ll dive into the physiological training adaptation mechanisms
, explore factors influencing your response, compare periodization approaches including the fitness fatigue model
, detail evidence based recovery strategies
, and discuss how to monitor your progress to truly optimize training for peak performance
.
Whether you’re a seasoned ultra runner or just starting to explore the science of endurance, understanding this principle is key. Let’s begin by defining supercompensation…
Chapter 1: Introduction: Understanding Supercompensation
1.1. Defining Supercompensation: The Core Principle of Adaptation
At the heart of all effective training lies a fundamental biological principle: supercompensation. It describes the body’s remarkable ability not just to recover from the stress of exercise, but to adapt and become stronger, faster, or more resilient than before.
Think of it as the body’s adaptive response to a challenge. When we apply a sufficient training stimulus – be it lifting weights, running sprints, or cycling long distances – we temporarily disrupt the body’s internal balance, or homeostasis. Supercompensation is the process that follows, where the body rebuilds itself to a level slightly above its previous baseline, preparing it to better handle similar stress in the future.
This “overshooting” of the baseline is the key to long-term progress in any athletic endeavor. Without understanding and applying this principle, training can become ineffective or even counterproductive.
1.2. Historical Context: From GAS to Modern Training Theory
The concept of adaptation to stress isn’t new. Early ideas can be traced back to Hans Selye’s work on the General Adaptation Syndrome (GAS) in the mid-20th century. Selye observed a general pattern in how organisms respond to various stressors, involving stages of alarm, resistance, and eventually exhaustion if the stress persists.
While GAS described a general stress response, exercise scientists like Yakovlev later applied similar concepts specifically to athletic training. They began to model how the body recovers and adapts following the specific stressor of exercise, laying the groundwork for the supercompensation theories used today.
These foundational ideas helped shift the focus from simply exercising to strategically planning training and recovery to maximize positive adaptations.
1.3. The Classic Four-Stage Model: Stimulus, Fatigue, Recovery, Adaptation
The supercompensation process is often visualized as a cyclical model involving four distinct phases:
- Stimulus (Training Stress): An exercise session of appropriate intensity and duration is applied, disrupting homeostasis and causing fatigue.
- Recovery (Compensation): The body begins repairing damage and replenishing depleted energy stores, aiming to return to its baseline state.
- Supercompensation (Rebound): If recovery is adequate, the body temporarily adapts beyond its initial baseline level, enhancing its capacity. This is the target phase for performance improvements.
- Involution (Detraining/Decline): If no further stimulus is applied during the supercompensation phase, or if the next stimulus is too weak, the adaptive gains gradually diminish, and the body returns towards its original baseline.
This simple model provides a useful framework for understanding the basic relationship between training, recovery, and adaptation. However, as we will explore later, the reality is more complex.
1.4. Why Supercompensation Matters: Optimizing Performance and Training
Understanding supercompensation is critical for anyone serious about improving athletic performance or physical fitness. It forms the basis for effective training program design.
The primary goal of training is not just to work hard, but to elicit specific, desirable adaptations that enhance physical work capacity. By manipulating training variables (like intensity, volume, and frequency) and managing recovery, coaches and athletes aim to consistently trigger the supercompensation response.
Mastering this process allows for:
- Progressive overload and long-term improvement.
- Peaking performance for specific competitions.
- Avoiding plateaus and overtraining.
In essence, supercompensation is the mechanism through which well-structured training translates into tangible results.
1.5. Purpose, Scope, and Structure of This Book
This book aims to provide a comprehensive yet accessible overview of the science behind supercompensation. We will delve deeper than the classic four-stage model, exploring the underlying physiological mechanisms, the factors that influence the adaptive response, and practical strategies for applying these principles.
We will examine:
- The molecular and cellular events driving adaptation (Chapter 2).
- How training variables and recovery factors modulate the response (Chapter 3).
- The application of supercompensation in periodization and tapering (Chapter 4).
- Evidence-based recovery strategies to enhance adaptation (Chapter 5).
- Limitations of the classic model and alternative perspectives (Chapter 6).
- The importance of monitoring and individualization (Chapter 7).
Our goal is to equip coaches, athletes, students, and practitioners with the knowledge to design more effective training programs based on a solid understanding of how the body adapts to exercise stress.
Chapter 2: The Physiological Engine: Mechanisms Driving Supercompensation
Supercompensation isn’t magic; it’s the result of complex, coordinated physiological processes occurring within the body in response to exercise stress. Understanding these underlying mechanisms helps us appreciate how training drives adaptation. This chapter explores the key cellular and molecular events that power recovery and the adaptive rebound.
2.1. Energy Store Dynamics: The Role of Glycogen
Muscle glycogen, the stored form of carbohydrates within muscle cells, is a primary fuel source, especially during moderate-to-high intensity exercise. Its depletion is a significant factor contributing to fatigue.
2.1.1. Post-Exercise Glycogen Resynthesis
Following exercise that depletes glycogen stores, the body initiates a process to replenish them. This resynthesis typically occurs in two phases: an initial rapid phase lasting a few hours (highly dependent on immediate carbohydrate intake) followed by a slower, more prolonged phase.
The rate and extent of glycogen depletion depend heavily on exercise intensity and duration. Intense activities can rapidly reduce glycogen levels, creating a powerful stimulus for its subsequent resynthesis and potential supercompensation.
2.1.2. Glycogen Supercompensation: More Particles, Not Bigger Ones?
When sufficient carbohydrates are consumed after glycogen-depleting exercise, muscles can store glycogen at levels exceeding their pre-exercise baseline – this is glycogen supercompensation. It’s a well-documented phenomenon, particularly relevant for endurance athletes.
Intriguingly, research suggests this increase in stored glycogen isn’t primarily due to existing glycogen particles getting larger. Instead, evidence points towards an increase in the number of glycogen particles within the muscle fibers, especially within Type I (slow-twitch) fibers after endurance exercise [source: 22]. This strategy might allow the muscle to store more fuel while maintaining rapid access to it when needed.
2.1.3. Molecular Drivers: The Roles of AMPK and Glycogen Synthase
Several key molecules regulate glycogen storage. Exercise activates AMP-activated protein kinase (AMPK), an energy sensor that promotes glucose uptake into the muscle. Simultaneously, the activity of Glycogen Synthase (GS), the enzyme responsible for building glycogen chains, is increased.
Studies indicate that the activation of AMPK and GS can persist even after glycogen levels have returned to normal following exercise [source: 25, 30]. This sustained molecular drive is thought to be crucial for overcoming the normal feedback mechanisms that would limit glycogen storage, allowing the muscle to achieve supercompensated levels.
2.2. Building and Repairing: Muscle Protein Synthesis (MPS)
Exercise, particularly resistance training, causes micro-damage to muscle fibers. The repair and rebuilding process, driven by Muscle Protein Synthesis (MPS), is fundamental to muscle adaptation, including increases in size (hypertrophy) and strength.
2.2.1. The Balance: MPS vs. Muscle Protein Breakdown (MPB)
Muscle tissue is constantly being remodeled through the competing processes of MPS (building) and Muscle Protein Breakdown (MPB; degrading). Net muscle growth occurs when the rate of MPS exceeds the rate of MPB over time.
Exercise stimulates both MPS and MPB. Consuming protein, especially essential amino acids, after exercise significantly boosts MPS while helping to suppress MPB, tipping the balance towards net protein gain and adaptation.
2.2.2. The “Muscle-Full” Concept and the Extended Anabolic Window
Traditionally, much emphasis was placed on a narrow “anabolic window” immediately post-exercise for protein intake. While consuming protein soon after training is beneficial, research has refined this concept.
Studies suggest a “muscle-full” effect, where a single dose of protein stimulates MPS for a limited time (perhaps 1.5-2 hours) before the muscle becomes temporarily refractory to further stimulation, even if amino acids are available [source: 31]. However, the crucial finding is that exercise significantly extends the period during which the muscle remains sensitized to amino acids, potentially lasting 24 hours or more [source: 31]. This highlights the importance of sufficient total daily protein intake, distributed throughout the day, in addition to post-exercise timing.
2.2.3. Specificity: Myofibrillar vs. Sarcoplasmic Protein Synthesis
Not all muscle protein synthesis is the same. Adaptations are specific to the type of training. Resistance exercise preferentially stimulates the synthesis of myofibrillar proteins – the actual contractile elements responsible for force production, leading to hypertrophy and strength gains [source: 31]. Endurance exercise tends to stimulate the synthesis of mitochondrial and sarcoplasmic proteins to a greater degree.
2.3. Cellular Control and Adaptation: Key Signaling Pathways
The adaptive responses to training are orchestrated by complex intracellular signaling networks that sense the exercise stress and trigger downstream changes in gene expression and protein synthesis.
2.3.1. Endurance Adaptations: Mitochondrial Biogenesis (PGC-1α)
Endurance training is renowned for increasing the muscle’s oxidative capacity – its ability to use oxygen to produce energy. A key adaptation is mitochondrial biogenesis, the creation of new mitochondria.
This process is largely governed by the master regulator PGC-1α (Peroxisome proliferator-activated receptor gamma coactivator 1-alpha). PGC-1α activity is increased by endurance exercise signals (like elevated calcium and AMPK activation), leading to enhanced mitochondrial content and function.
2.3.2. The AMPK-mTOR Axis: A Master Switch for Adaptation?
Two central signaling pathways often seen as opposing forces are AMPK and mTOR (mammalian Target of Rapamycin).
- AMPK: Activated by energy stress (common in endurance exercise), it promotes catabolic processes (like fat burning) and mitochondrial biogenesis while generally inhibiting mTOR and protein synthesis during exercise [source: 7, 39].
- mTOR: Activated by growth signals like amino acids, insulin, and mechanical load (especially from resistance exercise), it drives protein synthesis and muscle growth [source: 7, 41].
The interplay and balance between these pathways heavily influence whether the primary adaptation leans towards endurance (AMPK-driven) or strength/hypertrophy (mTOR-driven).
2.3.3. Understanding the Interference Effect
The potential conflict between AMPK and mTOR signaling provides a molecular basis for the “interference effect” sometimes observed during concurrent training (combining endurance and strength training in the same program). High volumes of endurance exercise might activate AMPK strongly enough to potentially blunt the mTOR signaling needed for maximal strength and hypertrophy adaptations from resistance training [source: 7]. However, the extent and practical significance of this interference depend on many factors, including training volume, intensity, and timing.
2.3.4. Other Modulating Pathways
Beyond AMPK and mTOR, numerous other signaling molecules and pathways (involving calcium, reactive oxygen species (ROS), p38 MAPK, sirtuins, etc.) contribute to the intricate regulation of exercise adaptation, fine-tuning the cellular response to different types of training stress [source: 43, 38].
Chapter 3: Tuning the Response: Factors Influencing Supercompensation
The supercompensation response isn’t a fixed, predictable event. Its magnitude, timing, and even whether it occurs at all are influenced by a multitude of interacting factors. Understanding these factors allows coaches and athletes to fine-tune training and recovery to maximize positive adaptations. This chapter explores the key variables that modulate the supercompensation curve.
3.1. The Training Stimulus: Dose-Response Relationship
The nature of the training stress itself is the primary driver initiating the fatigue-recovery-adaptation cycle. The “dose” of training significantly impacts the “response.”
3.1.1. Intensity: The Quality of Stress
Training intensity refers to how hard the exercise is performed (e.g., percentage of maximum heart rate, weight lifted relative to maximum). Higher intensity workouts generally impose greater physiological stress, requiring longer recovery periods [source: 10]. However, intensity is crucial for triggering specific adaptations, such as improvements in maximal strength or aerobic power [source: 37, 31]. Finding the right intensity is key to providing an effective stimulus without overwhelming the body’s recovery capacity.
3.1.2. Volume: The Quantity of Stress
Volume typically refers to the total amount of work performed (e.g., total distance run, total weight lifted – sets x reps x weight). Higher training volumes generally lead to greater fatigue and necessitate longer recovery times [source: 43]. While a certain volume is needed for adaptation, excessive volume can hinder recovery and blunt the supercompensation effect. Interestingly, in some contexts like team sports or initial training phases, lower volumes may yield similar fitness gains as higher volumes, suggesting a point of diminishing returns [source: 45].
3.1.3. Frequency: Timing the Next Bout
Frequency refers to how often training sessions are performed. Applying the next training stimulus too soon, before adequate recovery and supercompensation have occurred, can lead to accumulating fatigue and maladaptation. Conversely, waiting too long can mean missing the peak supercompensation window, resulting in lost adaptation potential [source: 1]. Optimal frequency depends on the interplay between training intensity, volume, and individual recovery capacity.
3.1.4. Training to Failure: Benefits and Drawbacks
In resistance training, performing sets to momentary muscular failure can be a potent stimulus for muscle growth. However, training to failure consistently increases perceived exertion and significantly prolongs recovery time compared to stopping sets short of failure, even when total volume is matched [source: 44, 49]. While it can be used strategically, frequent training to failure requires careful planning to allow sufficient recovery between sessions targeting similar muscle groups [source: 44].
3.1.5. Exercise Selection Matters
Different types of exercises impose varying levels of stress and require different recovery periods. Large compound movements involving multiple joints (like squats or deadlifts), exercises emphasizing the eccentric (lowering) phase, and exercises targeting large muscle groups generally induce more fatigue and require longer recovery than isolation exercises or those focusing on smaller muscle groups [source: 44, 49].
3.1.6. Load Management: The Acute:Chronic Workload Ratio (ACWR)
A popular concept for managing training load and injury risk is the ACWR. It compares the recent training load (acute load, typically over one week) to the longer-term average load (chronic load, typically over four weeks) [source: 11]. Rapid spikes in the acute load relative to the chronic load (a high ACWR) are associated with increased fatigue and injury risk, potentially disrupting the optimal supercompensation cycle. Maintaining the ACWR within a suggested “sweet spot” is thought to promote adaptation while mitigating risk.
3.2. Recovery: The Foundation for Adaptation
The training stimulus only provides the potential for adaptation; actual supercompensation occurs during the recovery period. Inadequate recovery negates the benefits of even the best training program.
3.2.1. Time: How Much Rest is Enough?
Sufficient time between stressful training sessions is paramount. The required duration depends on the nature of the training stimulus (intensity, volume, type) and individual factors. As discussed, higher intensity generally necessitates more recovery time [source: 10]. Monitoring the athlete’s response is crucial for determining adequate rest periods.
3.2.2. Nutrition: The 4 R’s Framework
Optimal nutrition is fundamental to recovery and adaptation. The “4 R’s” provide a useful framework [source: 48, 51]:
- Rehydrate: Replenish fluids and electrolytes lost through sweat.
- Refuel: Restore depleted muscle glycogen stores with adequate carbohydrate intake, timed appropriately.
- Repair: Consume sufficient high-quality protein to support muscle protein synthesis and tissue repair.
- Rest: While often linked to sleep, this also implies overall relaxation and avoiding further significant stress immediately post-exercise.
Failure to address these nutritional components can significantly impair recovery and limit supercompensation.
3.2.3. Sleep: The Ultimate Recovery Tool
Sleep is arguably the most critical recovery modality. During sleep, numerous restorative processes occur, including hormone release (like growth hormone), protein synthesis, and cognitive restoration [source: 11, 49]. Chronic sleep deprivation or poor sleep quality severely hampers recovery, increases fatigue, impairs performance, elevates injury risk, and disrupts the hormonal environment needed for adaptation [source: 49, 50]. Prioritizing sufficient, high-quality sleep is non-negotiable.
3.2.4. Life Stress: The Hidden Variable
The body doesn’t differentiate between training stress and other life stressors (work, relationships, finances, etc.). High levels of psychological or emotional stress activate similar physiological pathways as physical stress, consuming recovery resources [source: 4]. An athlete experiencing high life stress will have a reduced capacity to tolerate and adapt positively to training stress. Managing overall stress is therefore important for optimizing supercompensation.
Monitoring tools like perceived exertion (RPE), wellness questionnaires, and heart rate variability (HRV) can help gauge an athlete’s overall stress and recovery status [source: 11, 52].
3.3. Individual Variability: Why One Size Doesn’t Fit All
Athletes respond differently to the same training and recovery protocols. What works optimally for one individual may be suboptimal or even detrimental for another.
3.3.1. Training Status and Experience
Untrained individuals typically adapt more rapidly initially but may also experience more muscle damage and require longer recovery from novel stimuli. Highly trained athletes often recover faster from familiar workloads but require stronger or more specific stimuli to continue adapting [source: 10, 53]. Their window for supercompensation might also be narrower.
3.3.2. Age-Related Considerations
Age can influence both the adaptive response and recovery capacity. While older adults still benefit immensely from exercise, they may experience slightly different adaptation timelines or require adjustments in training volume or recovery periods compared to younger individuals [source: 53, 32, 43].
3.3.3. The Genetic Influence: Responders and Non-Responders?
Genetics plays a significant role in determining an individual’s inherent potential and response to training [source: 58, 59]. Variations (polymorphisms) in specific genes related to muscle structure (e.g., ACTN3), energy metabolism, or cardiovascular function (e.g., ACE) can influence endurance capacity, power potential, susceptibility to injury, and the magnitude of adaptation to a given training program [source: 59, 60]. While genetic testing for athletic potential is still evolving, it underscores the biological basis for individual differences in supercompensation [source: 58].
Chapter 4: Structuring Training: Periodization and Supercompensation
Applying the supercompensation principle effectively requires looking beyond individual workouts. Periodization is the strategic, long-term planning and structuring of training to optimize adaptations and performance outcomes, primarily by managing the interplay between training stress and recovery over time.
4.1. The Rationale for Periodization: Managing Stress and Adaptation
Training constantly at the same high intensity or volume eventually leads to stagnation or overtraining. Periodization provides a framework for systematically varying training variables (like volume, intensity, and exercise type) across different phases or cycles [source: 62, 1].
The core goal is to continually provide an effective training stimulus to drive adaptation while strategically incorporating periods of lower stress or active recovery to allow supercompensation to occur and mitigate excessive fatigue [source: 1, 66]. It’s about intelligently orchestrating the stress-recovery cycle over weeks, months, and even years.
4.2. Traditional Models: Linear vs. Undulating Periodization
Two foundational periodization models offer different approaches to varying the training load:
4.2.1. Linear (Classic) Periodization
The linear model typically involves progressing through distinct training phases (mesocycles), each focusing on a specific physical quality. A common pattern starts with higher volume and lower intensity (e.g., focusing on hypertrophy or anatomical adaptation) and gradually transitions towards lower volume and higher intensity (e.g., focusing on strength, power, or sport-specific skills) as a competition approaches [source: 62, 63].
This model aims to build a broad fitness base and then sharpen specific qualities, culminating in a planned peak (supercompensation) after a final tapering phase reduces accumulated fatigue [source: 63]. Its structured progression can be relatively simple to implement, especially for novice athletes.
4.2.2. Undulating Periodization (WUP/DUP)
Undulating or non-linear periodization involves more frequent variations in volume and intensity, often within the same training week (Weekly Undulating Periodization – WUP) or even daily (Daily Undulating Periodization – DUP) [source: 62, 64]. For example, a week might include separate days focused on hypertrophy, strength, and power.
The rationale is that frequent changes provide varied stimuli, potentially preventing accommodation and managing fatigue more effectively by not constantly overloading the same physiological systems [source: 64]. This approach might allow for more frequent opportunities for smaller supercompensation effects and is often considered effective for intermediate and advanced athletes who can tolerate varied stress [source: 65].
4.2.3. Model Comparison: Which is Superior?
Research comparing linear and undulating models has yielded mixed results, with no single model proving definitively superior in all situations [source: 62, 65]. Both periodized approaches generally outperform non-periodized training [source: 12].
Undulating models might show slightly better results in some studies, potentially due to the varied stimulus or shorter study durations not fully capturing the delayed peak of linear models [source: 62]. Ultimately, the best model depends on the individual athlete, their training history, the specific sport demands, and the timeframe.
4.3. Block Periodization: Concentrated Loading
Block Periodization (BP) offers an alternative structure, particularly popular among high-level athletes. It involves organizing training into specialized blocks (mesocycles), each focusing intensely on developing a minimal number of specific physical abilities [source: 67, 62].
4.3.1. The ATR Model (Accumulation, Transformation, Realization)
A common BP framework is the ATR model [source: 67]:
- Accumulation: Focuses on developing basic abilities, often involving high training volumes (e.g., general endurance, basic strength).
- Transformation: Concentrates on sport-specific abilities, typically involving higher intensity and reduced volume (e.g., specific endurance, maximal strength, power).
- Realization: A pre-competition phase focused on recovery, tapering, and maximizing preparedness for peak performance.
4.3.2. Rationale and Residual Training Effects
The core idea behind BP is to provide a highly concentrated training stimulus for a specific ability within each block, aiming to maximize adaptation in that quality [source: 70, 71]. This intense focus potentially leads to significant fatigue within the block, followed by recovery and supercompensation for that specific ability during the subsequent, less demanding block [source: 72, 73].
BP relies on the concept of residual training effects – the idea that adaptations gained in one block persist (for varying durations depending on the quality) and support the development of abilities in the next block [source: 69]. This sequential, concentrated approach aims to achieve higher peaks in key abilities compared to trying to develop many qualities simultaneously [source: 74].
4.4. Peaking for Performance: Tapering Strategies
Regardless of the periodization model used, tapering is a critical strategy employed before major competitions to achieve peak performance [source: 76]. It involves a planned reduction in training load to allow accumulated fatigue to dissipate while maintaining or even enhancing fitness adaptations [source: 76, 4].
4.4.1. The Science of Tapering: Reducing Fatigue, Maximizing Fitness
Tapering works because fatigue generally dissipates faster than fitness declines [source: 1, 4]. By significantly reducing the training stress (primarily volume), the athlete sheds accumulated fatigue, allowing the underlying fitness gains (built up during harder training phases) to fully manifest as improved performance – effectively timing the supercompensation peak [source: 76].
4.4.2. Manipulating Volume, Intensity, and Frequency
The most crucial element of a successful taper is a substantial reduction in training volume (often 40-70% or more) [source: 76, 45]. It is generally recommended to maintain training intensity close to pre-taper levels to preserve fitness adaptations. Training frequency may be maintained or slightly reduced [source: 76]. Reducing volume too little or dropping intensity too much can lead to an ineffective taper.
4.4.3. Types and Duration of Tapers
Tapers can follow different patterns of load reduction (e.g., linear, step, exponential/progressive), with evidence suggesting exponential tapers (where the load reduction is greatest initially) may be particularly effective [source: 77, 45]. The optimal taper duration varies depending on the sport, the preceding training load, and the individual, but typically ranges from a few days to 2-3 weeks [source: 77, 52]. A well-executed taper can lead to significant performance improvements.
Chapter 5: Enhancing Adaptation: Evidence-Based Recovery Strategies
While training provides the stimulus for adaptation, recovery is where the magic happens. Implementing effective recovery strategies can accelerate the repair process, reduce fatigue, and potentially enhance the supercompensation effect, allowing athletes to train more effectively and consistently. This chapter examines various recovery modalities, focusing on the scientific evidence supporting their use.
5.1. Nutritional Interventions Revisited
As discussed in Chapter 3, nutrition forms the bedrock of recovery. Optimizing nutrient intake and timing is crucial for replenishing energy stores and repairing damaged tissues [source: 81].
5.1.1. Optimizing Macronutrient Timing and Intake
Following the “4 R’s” framework (Rehydrate, Refuel, Repair, Rest) provides practical guidance [source: 48, 81]:
- Rehydrate: Focus on replacing fluid losses, aiming for approximately 1.5 liters of fluid for every kilogram of body weight lost during exercise, especially if the next session is soon. Including electrolytes like sodium can aid retention [source: 83, 84].
- Refuel: Prioritize carbohydrate intake soon after exercise (e.g., 1.0-1.2 g/kg/hour) to maximize glycogen resynthesis rates, especially during the initial rapid phase [source: 85, 20]. High-glycemic index sources may be beneficial initially [source: 29].
- Repair: Consume high-quality protein (e.g., 0.3 g/kg or 20-40g absolute, rich in leucine) post-exercise to stimulate MPS. Distributing protein intake throughout the day is also important [source: 85, 26, 36].
- Rest (Nutritional Context): Consuming protein, particularly slow-digesting casein (around 40g), before sleep may enhance overnight MPS and support recovery [source: 86, 48].
5.1.2. Evidence for Recovery Supplements
Beyond macronutrients, certain supplements have garnered attention for their potential recovery benefits. It’s crucial to evaluate these based on scientific evidence:
- Strong Evidence:
- Tart Cherry Juice/Concentrate: Rich in polyphenols with anti-inflammatory and antioxidant properties. Consistently shown to reduce muscle soreness (DOMS), decrease inflammation markers, and accelerate the recovery of muscle strength and function [source: 87, 145, 36].
- Omega-3 Fatty Acids (EPA/DHA): Possess anti-inflammatory effects. Regular intake may help reduce muscle soreness, support muscle repair, and potentially improve muscle function after damaging exercise [source: 87, 145, 36].
- Moderate Evidence:
- Creatine Monohydrate: Primarily known for enhancing high-intensity performance, creatine may also aid recovery by reducing exercise-induced inflammation and potentially enhancing glycogen resynthesis when co-ingested with carbohydrates [source: 88, 28].
- Vitamin D: May play a role in muscle repair and immune function, potentially supporting recovery, especially in individuals with deficiency.
- Limited/Emerging Evidence: Compounds like collagen/gelatin (for connective tissue), curcumin, and bromelain show some potential but require more robust research regarding recovery benefits [source: 88, 36]. Routine high-dose antioxidant supplementation is generally discouraged; focus on whole food sources [source: 88].
5.2. Active vs. Passive Recovery: Which is Better, and When?
Recovery between exercise bouts or immediately post-session can be active (low-intensity movement) or passive (rest).
- Active Recovery (ACT): Involves light activity (e.g., easy cycling, jogging, swimming) typically below 60% VO2max [source: 88].
- Passive Recovery (PAS): Involves complete rest or inactivity [source: 88].
ACT demonstrably clears blood lactate faster than PAS [source: 88, 72]. It can also help lower heart rate and body temperature more quickly post-exercise [source: 72]. However, its impact on subsequent performance and long-term adaptation is less clear and appears highly context-dependent [source: 89, 90].
Some studies show ACT improves subsequent performance, while others find no difference [source: 89]. There’s also a theoretical concern: the metabolic stress (like lactate accumulation) that ACT helps clear might itself be an important signal for adaptation [source: 92, 74]. Reducing this signal via ACT could potentially blunt long-term adaptations from certain types of training (like HIIT), although research here is conflicting, with some studies showing no negative impact or even enhanced adaptation with ACT [source: 93, 71, 74].
Therefore, the choice between ACT and PAS should consider the specific goal (e.g., rapid lactate clearance for same-day competitions vs. maximizing adaptation signals) and the time available between sessions [source: 95].
5.3. Sleep Optimization Strategies for Athletes
As emphasized previously, sleep is paramount [source: 96, 51]. Strategies to optimize sleep include:
- Maintaining a consistent sleep-wake schedule, even on weekends.
- Creating a cool, dark, and quiet sleep environment.
- Avoiding excessive caffeine and alcohol, especially close to bedtime.
- Limiting screen time and blue light exposure before bed.
- Developing a relaxing pre-sleep routine.
- Considering naps strategically if overnight sleep is insufficient, but avoiding long/late naps that disrupt nighttime sleep.
Monitoring sleep duration and quality (subjectively or via wearables) can help identify issues impacting recovery [source: 11].
5.4. Other Modalities: Weighing the Evidence
Various other recovery tools are popular among athletes:
5.4.1. Compression Garments (CGs)
Wearing compression garments during or after exercise is common. Meta-analyses suggest CGs offer small but statistically significant benefits for recovery [source: 97, 77, 155]. They appear particularly consistent in reducing perceived muscle soreness (DOMS) [source: 97, 76]. Evidence also points towards aiding the recovery of muscle power after resistance exercise, especially beyond 24 hours [source: 97, 77]. The exact mechanisms are debated but may involve reduced muscle oscillation, altered blood flow, or reduced swelling. Comfort and appropriate pressure are important [source: 97, 98].
5.4.2. Cryotherapy (Cold Water Immersion / Whole Body Cryotherapy)
Exposing the body to cold (e.g., ice baths, whole-body cryotherapy – WBC) is another popular strategy aimed at reducing inflammation, swelling, and muscle soreness [source: 98, 61]. WBC involves brief exposure to extremely cold air.
Evidence suggests potential benefits for reducing DOMS and perceived fatigue [source: 61]. However, responses can be highly individual, influenced by factors like gender, body composition, and the specific protocol used [source: 99, 61]. A “one-size-fits-all” approach is ineffective and potentially unsafe [source: 99]. Furthermore, some research raises concerns that regular cold exposure might blunt long-term muscular adaptations (hypertrophy) by suppressing inflammatory signals necessary for remodeling.
5.4.3. Brief Overview: Massage, Stretching, Foam Rolling
Other common modalities include massage, stretching, and foam rolling. Massage likely aids recovery primarily through reducing perceived soreness and promoting relaxation. The benefits of static stretching for acute recovery are minimal and may even temporarily reduce force production. Foam rolling may acutely improve range of motion and reduce perceptions of muscle soreness, though its impact on objective recovery markers is less clear.
Ultimately, while various modalities exist, prioritizing foundational strategies like nutrition and sleep is likely the most effective approach to optimizing recovery and maximizing supercompensation.
Chapter 6: Critical Perspectives: Limitations and Alternative Models
While the classic supercompensation model provides a valuable conceptual framework for understanding training adaptation, it’s essential to recognize its limitations. The biological reality is far more complex than a simple four-stage curve suggests. This chapter critically examines the shortcomings of the traditional model and introduces an influential alternative: the Fitness-Fatigue model.
6.1. Questioning the Classic Model: Where Does it Fall Short?
Despite its intuitive appeal, the classic supercompensation model faces several criticisms when scrutinized against scientific evidence and practical application:
6.1.1. Lack of Universal Evidence and Generalization Issues
The model is often based on the well-documented supercompensation of muscle glycogen [source: 103]. However, evidence for a similar supercompensatory rebound is lacking for many other physiological markers or metabolites following exercise [source: 69, 160]. Generalizing the distinct glycogen response to encompass all aspects of training adaptation is questionable [source: 103].
6.1.2. The Problem of Timing and Differential Recovery
Different physiological systems recover at vastly different rates [source: 104, 1]. For example, neural recovery, muscle tissue repair, and glycogen replenishment follow distinct timelines. The classic model, with its single curve, fails to account for these varied recovery kinetics, making it difficult to determine the truly optimal time to apply the next training stimulus for all relevant systems simultaneously [source: 105, 69].
6.1.3. Oversimplification of a Complex Process
Representing adaptation as a single wave-like curve drastically oversimplifies the intricate interplay of physiological, biomechanical, and even psychological responses to training [source: 106, 1]. It doesn’t adequately capture the effects of different types of fatigue (e.g., central vs. peripheral) or the influence of non-training stressors [source: 106, 4]. Furthermore, it implies a purely sequential process, neglecting the reality that training simultaneously builds fitness and induces fatigue [source: 107].
6.1.4. Conflicts with Empirical Observations
Observed recovery and adaptation timelines sometimes contradict the model’s typical representation. For instance, studies examining neuromuscular function after heavy resistance training have shown recovery and even supercompensation occurring much faster (within hours) than the commonly suggested 24-48 hour timeframe for muscle recovery [source: 108, 109, 55, 163]. Additionally, the transient nature of peak performance (often lasting only days or a couple of weeks) contrasts with the potentially prolonged peak implied by a simple curve [source: 110, 67].
6.2. The Fitness-Fatigue Model: A More Dynamic View?
Recognizing the limitations of the classic model, researchers like Zatsiorsky and Banister proposed the Fitness-Fatigue (or Two-Factor) Model [source: 112, 1, 4]. This model is often considered a more sophisticated representation of the training response.
6.2.1. Core Concepts: Two Factors, Different Decay Rates
The central premise is that every training bout produces two concurrent after-effects [source: 112]:
- Fitness Effect: The positive adaptive outcome of the training stimulus. This effect is considered relatively long-lasting but moderate in magnitude [source: 113, 1].
- Fatigue Effect: The negative consequence of training, impairing performance. This effect is thought to be larger in magnitude initially but dissipates much more quickly than the fitness effect [source: 114, 1, 4].
An athlete’s preparedness or performance potential at any given time is the net result of these two opposing factors: Preparedness = Fitness – Fatigue [source: 114, 5].
6.2.2. Explaining Performance Fluctuations and Tapering
The Fitness-Fatigue model offers several advantages:
- It explicitly acknowledges the simultaneous positive (fitness) and negative (fatigue) consequences of training [source: 115, 5].
- It provides a clearer mechanistic explanation for tapering. By reducing training load (especially volume), fatigue input is minimized. Because fatigue dissipates faster than fitness, the net effect is a rapid decrease in fatigue, “unmasking” the accumulated fitness and leading to peak preparedness [source: 115, 116, 4].
- It helps explain why performance might temporarily decrease during hard training blocks (due to high fatigue accumulation masking fitness gains) and then rebound quickly with rest [source: 116].
- It incorporates both the magnitude (intensity) and duration (volume) of training stress in influencing both fitness and fatigue components [source: 4].
6.2.3. Comparing the Models: Complementary Perspectives
The Fitness-Fatigue model isn’t necessarily a replacement for the supercompensation concept but rather a more dynamic and detailed explanation of the underlying processes [source: 118, 170].
The classic supercompensation model remains a useful introductory tool for visualizing the basic principle of stress-recovery-adaptation [source: 1]. The Fitness-Fatigue model provides a more nuanced framework that better explains performance variations over time, particularly the effectiveness of tapering strategies [source: 184]. Both contribute to our understanding of how to structure training for optimal results.
Chapter 7: Monitoring and Individualization: Tailoring Training for Optimal Results
Previous chapters have highlighted the numerous factors influencing the supercompensation response – from training variables to genetics to sleep quality. This inherent complexity underscores a crucial point: effective training must be individualized. A one-size-fits-all program, even one based on sound physiological principles, will rarely yield optimal results for every athlete.
7.1. The Imperative of Individualization
Athletes differ significantly in their response to training loads and their recovery capacity [source: 185]. Factors like genetic predisposition [source: 58], training history [source: 53], age [source: 53], lifestyle stress [source: 52], and even daily fluctuations in readiness mean that a standardized approach is suboptimal.
The goal of individualization is to tailor the training process – the application of stress and the provision for recovery – to the specific needs and responses of the individual athlete. This requires moving beyond generic plans and implementing systems to monitor how the athlete is actually coping with and adapting to the training program.
7.2. The Coach’s and Athlete’s Toolkit: Monitoring Adaptation and Recovery
Monitoring provides objective and subjective data to track an athlete’s fatigue levels, recovery status, and readiness to train. This information is vital for making informed decisions about adjusting training loads and recovery strategies [source: 186]. Combining different tools often provides the most comprehensive picture.
7.2.1. Subjective Measures: Listening to the Athlete
Subjective feedback provides valuable insight into the athlete’s internal state and perception of effort and recovery:
- Rating of Perceived Exertion (RPE): A simple scale (e.g., 6-20 or 0-10) used during or after training to quantify the perceived difficulty of the session. Session RPE (sRPE), calculated by multiplying session duration by RPE, is a common way to estimate internal training load.
- Wellness Questionnaires: Short daily or regular questionnaires asking athletes to rate factors like fatigue, sleep quality, muscle soreness, stress levels, and mood [source: 11, 52]. Tools like RESTQ-Sport or simple custom questionnaires can track trends and flag potential issues [source: 166].
Consistent tracking of subjective measures can reveal patterns related to fatigue accumulation and recovery.
7.2.2. Objective Measures: Quantifying the Response
Objective data complements subjective feedback, providing physiological or performance-based insights:
- Heart Rate Variability (HRV): Measures the variation in time between consecutive heartbeats, reflecting the balance of the autonomic nervous system. Lower HRV can indicate increased physiological stress or incomplete recovery, while higher HRV generally suggests better readiness [source: 10, 11, 52]. Daily morning measurements are common.
- Performance Tests: Regularly assessing performance in key exercises or standardized tests (e.g., jump height, sprint times, submaximal heart rate during a set workload) can indicate changes in fitness or fatigue. A decline in performance can signal excessive fatigue.
- Training Load Monitoring: Tracking external load (e.g., weight lifted, distance covered, power output) and internal load (e.g., sRPE, heart rate response) provides data for calculating metrics like the Acute:Chronic Workload Ratio (ACWR) to manage load progression and injury risk [source: 11].
- Resting Heart Rate (RHR): While less sensitive than HRV, a consistently elevated RHR can sometimes indicate accumulated fatigue or inadequate recovery.
7.2.3. The Potential Role of Biomarkers
Research continues to explore the use of biomarkers (e.g., cortisol, creatine kinase, inflammatory markers in blood or saliva) to assess training stress and recovery. However, the cost, practicality, and day-to-day variability currently limit their widespread use in routine monitoring outside of specific research or elite settings.
7.3. Putting it Together: Using Data to Guide Training Decisions
Monitoring data is only useful if it informs action. The goal is to integrate information from various sources (subjective, objective, performance) to make timely adjustments to the training plan.
Examples of data-driven decisions include:
- Reducing training volume or intensity if multiple indicators (e.g., low HRV, high fatigue ratings, performance decline) suggest poor recovery.
- Increasing recovery interventions (e.g., prioritizing sleep, scheduling an active recovery day) if fatigue is accumulating.
- Progressing training load cautiously when monitoring suggests the athlete is coping well and adapting positively.
- Identifying individual patterns, such as how quickly an athlete typically recovers from specific types of workouts.
This feedback loop – Train -> Monitor -> Analyze -> Adjust -> Train – is central to optimizing the supercompensation process for each individual.
7.4. Practical Scenarios and Case Studies
Consider Athlete A, whose HRV drops significantly for two consecutive days alongside reports of poor sleep and high muscle soreness via a wellness questionnaire. This pattern might prompt their coach to replace a planned high-intensity session with active recovery or a lighter technique workout.
Conversely, Athlete B consistently shows good HRV scores, low RPEs relative to the external load, and positive wellness ratings during a training block. This data might support a decision to slightly increase the training volume or intensity in the following block, assuming continued positive adaptation.
By systematically monitoring and responding to individual feedback, training becomes a more precise and effective process, maximizing the potential for successful supercompensation.
Chapter 8: Conclusion: Principles into Practice
Our journey through the science of supercompensation has taken us from fundamental concepts to intricate molecular mechanisms, practical training structures, and evidence-based recovery strategies. We’ve seen that while the basic idea of stress, recovery, and adaptation is simple, optimizing this process requires a deep understanding of the underlying physiology and a nuanced, individualized approach.
8.1. Synthesizing the Science: Key Takeaways
Several core principles emerge from the evidence discussed throughout this book:
- Adaptation is Driven by Stress and Recovery: Supercompensation, or the positive adaptation described by the more dynamic Fitness-Fatigue model, is the desired outcome of training. It requires applying an appropriate training stimulus that disrupts homeostasis, followed by sufficient recovery to allow adaptation to occur [source: 168, 170].
- Mechanisms are Complex and Interconnected: Adaptations involve intricate changes in energy metabolism (glycogen storage dynamics [source: 171]), protein synthesis (MPS regulation, the “muscle-full” concept [source: 172]), and cellular signaling pathways (AMPK-mTOR balance, mitochondrial biogenesis [source: 173]).
- Numerous Factors Modulate the Response: The magnitude and timing of adaptation are influenced by training variables (intensity, volume, frequency, exercise choice [source: 174]), crucial recovery factors (nutrition, sleep, stress management [source: 174]), and significant individual variability (genetics, age, training status [source: 174, 175]).
- Structure and Planning are Essential: Periodization models (Linear, Undulating, Block) provide frameworks for strategically managing training stress and recovery over time to target specific adaptations and peak performance [source: 176, 177]. Tapering is a vital strategy to realize accumulated fitness gains by minimizing fatigue before key events [source: 178].
- Recovery Strategies Vary in Effectiveness: Foundational strategies like adequate nutrition (the 4 R’s) and sufficient sleep are paramount [source: 179, 180]. Other modalities like specific supplements (tart cherry, omega-3s), compression garments, or active recovery can offer context-dependent benefits, but their use should be guided by evidence [source: 181, 182].
- Individualization is Key: Due to the multitude of influencing factors, a one-size-fits-all approach is ineffective. Monitoring individual responses and adjusting training accordingly is crucial for optimizing adaptation [source: 175, 185].
8.2. Practical Recommendations for Athletes and Coaches
Translating these scientific principles into effective practice involves several key actions:
- Embrace Conceptual Models, But Flexibly: Use models like Supercompensation and Fitness-Fatigue as guiding frameworks, but understand their limitations and don’t apply them rigidly [source: 183, 184].
- Prioritize Foundational Recovery: Focus relentlessly on optimizing sleep quality and duration, and implementing sound nutritional strategies (adequate energy, carbohydrates, protein, hydration) before investing heavily in supplementary recovery modalities [source: 189, 190].
- Monitor the Individual Response: Implement a system for tracking both subjective (RPE, wellness) and objective (HRV, performance, load) indicators of the athlete’s response to training. Use this data to guide adjustments [source: 185, 186].
- Manipulate Training Variables Intelligently: Understand how changes in volume, intensity, frequency, and exercise selection impact stress and recovery requirements. Structure training using periodization principles appropriate for the athlete’s goals and level [source: 187, 188].
- Apply Evidence-Based Practices: When considering recovery strategies or supplements, evaluate the scientific evidence critically. Prioritize interventions with strong support for the desired outcome [source: 189].
- Link Theory to Practice: Continuously strive to understand the physiological reasons behind training methods and recovery strategies. This allows for more informed decision-making and problem-solving [source: 191].
8.3. Unanswered Questions and Future Directions
Despite significant advances, research into training adaptation continues. Areas for future exploration include further elucidating the complex interplay of signaling pathways, refining our understanding of individual genetic influences on trainability and recovery, optimizing nutritional strategies for specific contexts, and developing more practical and reliable real-time monitoring tools.
By integrating current scientific knowledge with careful monitoring and thoughtful application, coaches and athletes can navigate the complexities of the training process, effectively harness the power of supercompensation, and unlock greater levels of performance [source: 194].
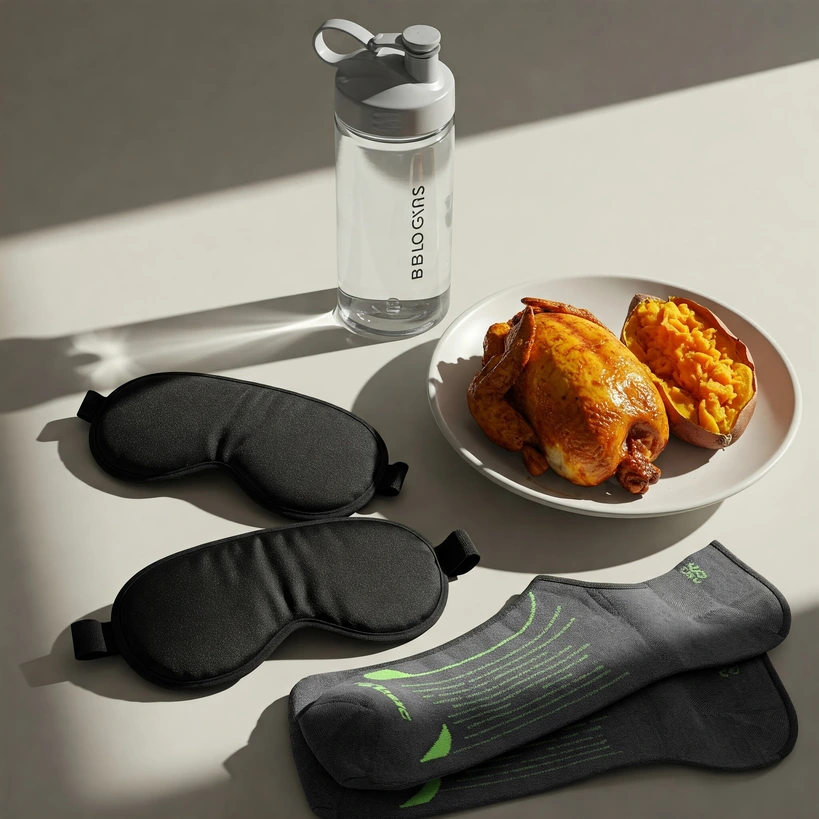
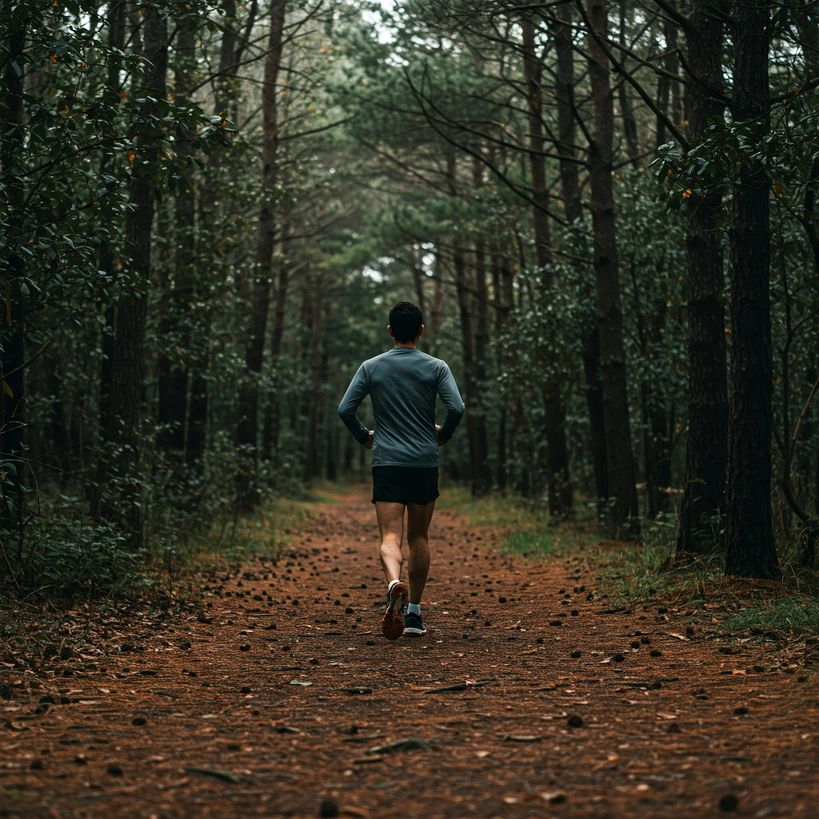
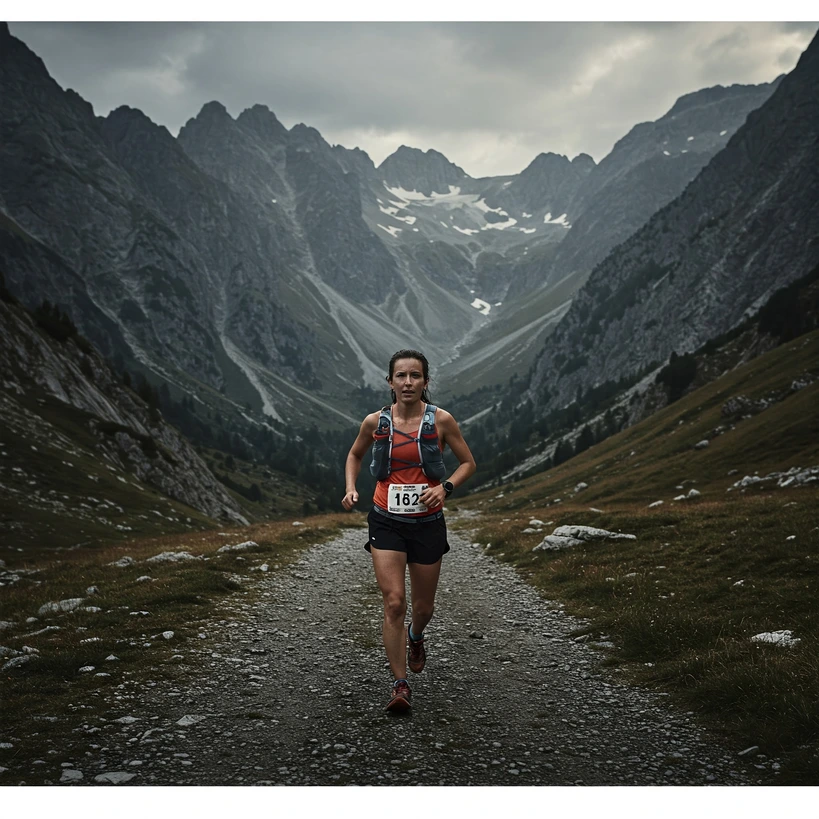
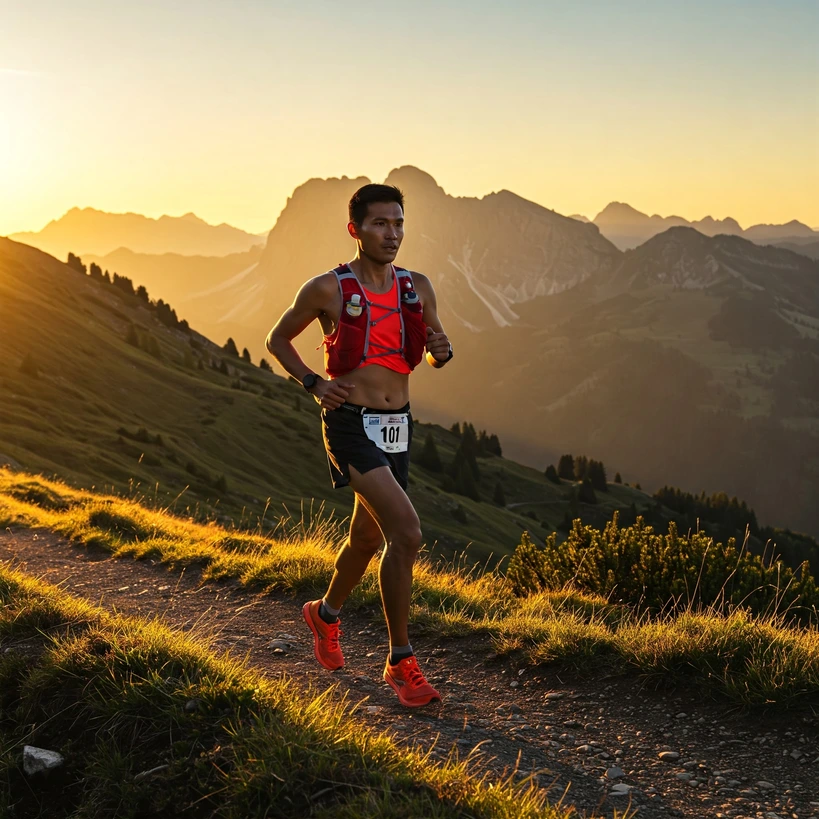
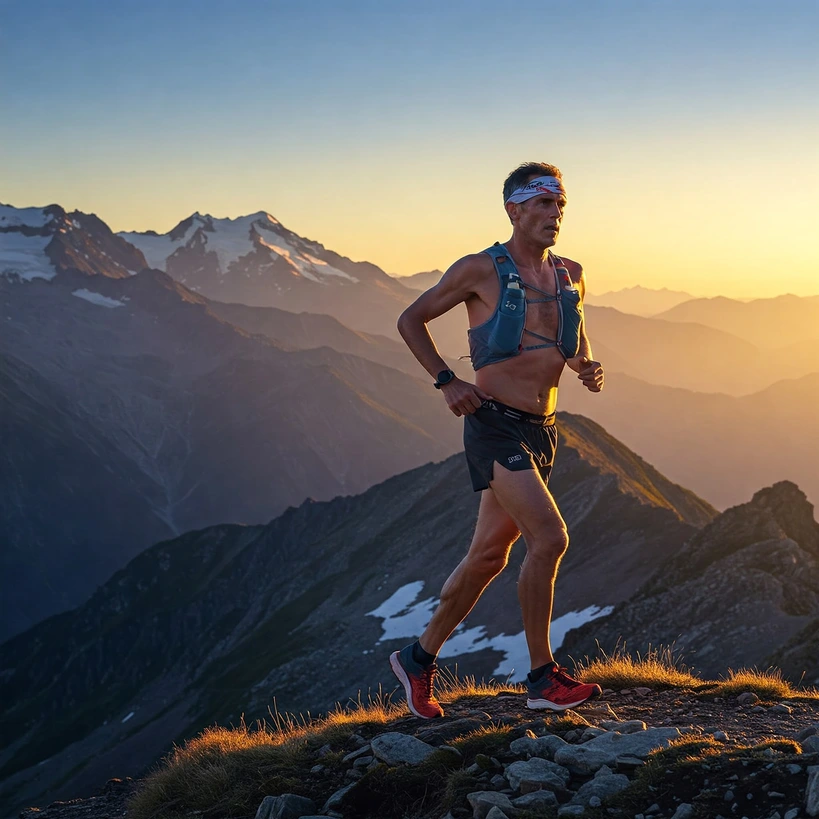
References
Key Foundational References
Below are a few selected references, particularly valuable for understanding the core concepts discussed in this book, with direct links for access:
-
Glycogen Supercompensation Mechanism (Particle Number):
Nielsen, J., et al. (2021). Glycogen supercompensation is due to increased number, not size, of glycogen particles in human skeletal muscle. Experimental Physiology, 106(5), 1197-1209.
PubMed Abstract/Link | DOI Link -
Glycogen Supercompensation Molecular Drivers (AMPK/GS):
Hostrup, M., et al. (2018). Molecular mechanisms promoting glycogen supercompensation in human skeletal muscle: roles of AMPK and glycogen synthase. Molecular Metabolism, 15, 16-28.
PubMed Link | DOI Link | Full Text (PMC) -
Muscle Protein Synthesis & “Muscle-Full” Concept:
Atherton, P. J., & Smith, K. (2012). Muscle protein synthesis in response to nutrition and exercise. The Journal of Physiology, 590(5), 1049-1057.
Full Text (PMC) | DOI Link -
HRV for Recovery Monitoring:
Stanley, J., et al. (2013). Cardiac parasympathetic reactivation following exercise: implications for training prescription. Sports Medicine, 43(12), 1363-1371.
PubMed Abstract/Link | DOI Link -
Periodization Concepts (Overview):
Cissik, J. M., et al. (2008). CURRENT CONCEPTS IN PERIODIZATION OF STRENGTH AND CONDITIONING FOR THE SPORTS PHYSICAL THERAPIST. North American Journal of Sports Physical Therapy: NAJSPT, 3(1), 53–69.
Full Text (PMC) -
Recovery Nutrition (4 R’s Framework):
Bonilla, D. A., et al. (2021). The 4R’s Framework of Nutritional Strategies for Post-Exercise Recovery: A Review with Emphasis on New Generation Ingredients. International Journal of Environmental Research and Public Health, 18(1), 103.
Full Text (PMC) | DOI Link -
Fitness-Fatigue Model & Tapering Explanation (Book Reference):
Zatsiorsky, V. M., & Kraemer, W. J. (2006). Science and Practice of Strength Training (2nd ed.). Human Kinetics. (Specifically Chapter 1 discusses relevant theories – direct online link may not be available, this is a book reference.)
Full Reference List
- Defining supercompensation training – Human Kinetics, access date April 21, 2025, https://us.humankinetics.com/blogs/excerpt/defining-supercompensation-training
- Physiological basis of adaptation through super-compensation for better sporting result, access date April 21, 2025, https://www.turkishkinesiology.com/index.php/ahe/article/view/13
- Physiological basis of adaptation through super-compensation for better sporting result – National Kinesiology, access date April 21, 2025, https://www.turkishkinesiology.com/index.php/ahe/article/download/13/12/58
- Training Adaptations—Fitness Fatigue Model – JoshStrength, access date April 21, 2025, https://joshstrength.com/2022/01/training-adaptations-fitness-fatigue-model/
- Hard Training + Efficient Recovery = Peak Performance | Performance Lab®, access date April 21, 2025, https://www.performancelab.com/blogs/fitness/hard-training-efficient-recovery-peak-performance
- Central Concepts Related to Periodization – NSCA, access date April 21, 2025, https://www.nsca.com/education/articles/kinetic-select/central-concepts-related-to-periodization/
- A Brief Review on Concurrent Training: From Laboratory to the Field – MDPI, access date April 21, 2025, https://www.mdpi.com/2075-4663/6/4/127
- Supercompensation: What it is, How it Works, and When to Use It – Hevy Coach, access date April 21, 2025, https://hevycoach.com/glossary/supercompensation/
- What is Supercompensation Theory and Why Should You Care? – Team Wilpers, access date April 21, 2025, https://blog.teamwilpers.com/what-is-supercompensation-theory-and-why-should-you-care/
- Cardiac parasympathetic reactivation following exercise … – PubMed, access date April 21, 2025, https://pubmed.ncbi.nlm.nih.gov/23912805/
- Monitoring training load and recovery | Exercise Physiology Class Notes – Fiveable, access date April 21, 2025, https://library.fiveable.me/exercise-physiology/unit-13/monitoring-training-load-recovery/study-guide/s3WZ7NdricDsF6Re
- CURRENT CONCEPTS IN PERIODIZATION OF STRENGTH AND CONDITIONING FOR THE SPORTS PHYSICAL THERAPIST – PMC – PubMed Central, access date April 21, 2025, https://pmc.ncbi.nlm.nih.gov/articles/PMC4637911/
- Recovery Methods in Basketball: A Systematic Review – PMC – PubMed Central, access date April 21, 2025, https://pmc.ncbi.nlm.nih.gov/articles/PMC10675622/
- Impact of Resistance Training on Sports Performance and Muscular Adaptations, access date April 21, 2025, https://clinmedjournals.org/articles/ijsem/international-journal-of-sports-and-exercise-medicine-ijsem-8-218.php?jid=ijsem
- Nutrition and muscle protein synthesis: a descriptive review – PMC – PubMed Central, access date April 21, 2025, https://pmc.ncbi.nlm.nih.gov/articles/PMC2732256/
- Exercise Load Recovery Assessment – Frontiers, access date April 21, 2025, https://www.frontiersin.org/journals/sports-and-active-living/articles/10.3389/fspor.2020.00067/epub
- Weekly Time Course of Neuro-Muscular Adaptation to … – Frontiers, access date April 21, 2025, https://www.frontiersin.org/journals/physiology/articles/10.3389/fphys.2017.00329/full
- Nutrient timing revisited: is there a post-exercise anabolic window? – PMC – PubMed Central, access date April 21, 2025, https://pmc.ncbi.nlm.nih.gov/articles/PMC3577439/
- Regulation of Muscle Glycogen Metabolism during Exercise: Implications for Endurance Performance and Training Adaptations – PMC – PubMed Central, access date April 21, 2025, https://pmc.ncbi.nlm.nih.gov/articles/PMC5872716/
- Restoration of Muscle Glycogen and Functional Capacity: Role of Post-Exercise Carbohydrate and Protein Co-Ingestion – PubMed Central, access date April 21, 2025, https://pmc.ncbi.nlm.nih.gov/articles/PMC5852829/
- Postexercise muscle glycogen resynthesis in humans | Journal of Applied Physiology, access date April 21, 2025, https://journals.physiology.org/doi/10.1152/japplphysiol.00860.2016
- Glycogen supercompensation is due to increased number, not size …, access date April 21, 2025, https://pubmed.ncbi.nlm.nih.gov/33675088/
- Effects of depletion exercise and light training on muscle glycogen supercompensation in men – PubMed, access date April 21, 2025, https://pubmed.ncbi.nlm.nih.gov/12902321/
- Persistence of supercompensated muscle glycogen in trained subjects after carbohydrate loading – PubMed, access date April 21, 2025, https://pubmed.ncbi.nlm.nih.gov/9029236/
- Exercise-induced molecular mechanisms promoting glycogen supercompensation in human skeletal muscle – PubMed, access date April 21, 2025, https://pubmed.ncbi.nlm.nih.gov/30093357/
- Nutrition and Supplement Update for the Endurance Athlete: Review and Recommendations – PMC – PubMed Central, access date April 21, 2025, https://pmc.ncbi.nlm.nih.gov/articles/PMC6628334/
- Fundamentals of glycogen metabolism for coaches and athletes – PMC – PubMed Central, access date April 21, 2025, https://pmc.ncbi.nlm.nih.gov/articles/PMC6019055/
- International Society of Sports Nutrition position stand: Nutrient timing – PMC – PubMed Central, access date April 21, 2025, https://pmc.ncbi.nlm.nih.gov/articles/PMC2575187/
- Carbohydrates and Endurance Exercise: A Narrative Review of a Food First Approach, access date April 21, 2025, https://pmc.ncbi.nlm.nih.gov/articles/PMC10054587/
- Exercise-induced molecular mechanisms promoting glycogen …, access date April 21, 2025, https://pmc.ncbi.nlm.nih.gov/articles/PMC6158101/
- Muscle protein synthesis in response to nutrition and exercise – PMC, access date April 21, 2025, https://pmc.ncbi.nlm.nih.gov/articles/PMC3381813/
- The Interplay Between Physical Activity, Protein Consumption, and Sleep Quality in Muscle Protein Synthesis – arXiv, access date April 21, 2025, https://arxiv.org/html/2410.16169v1
- Potential mechanisms involved in regulating muscle protein turnover after acute exercise: A brief review – Frontiers, access date April 21, 2025, https://www.frontiersin.org/journals/physiology/articles/10.3389/fphys.2022.1106425/full
- Full article: Protein for adaptations to exercise training – Taylor & Francis Online, access date April 21, 2025, https://www.tandfonline.com/doi/full/10.1080/17461390801919102
- Maximizing Post-exercise Anabolism: The Case for Relative Protein Intakes – Frontiers, access date April 21, 2025, https://www.frontiersin.org/journals/nutrition/articles/10.3389/fnut.2019.00147/full
- Selected In-Season Nutritional Strategies to Enhance Recovery for Team Sport Athletes: A Practical Overview – PubMed Central, access date April 21, 2025, https://pmc.ncbi.nlm.nih.gov/articles/PMC5633631/
- Adaptations to Endurance and Strength Training – PMC, access date April 21, 2025, https://pmc.ncbi.nlm.nih.gov/articles/PMC5983157/
- The molecular athlete: exercise physiology from mechanisms to medals – PubMed Central, access date April 21, 2025, https://pmc.ncbi.nlm.nih.gov/articles/PMC10110736/
- AMPK in skeletal muscle function and metabolism – PMC, access date April 21, 2025, https://pmc.ncbi.nlm.nih.gov/articles/PMC5945561/
- Mechanical Loading Modulates AMPK and mTOR Signaling in Muscle Cells – PubMed, access date April 21, 2025, https://pubmed.ncbi.nlm.nih.gov/39213513/
- Regulation of mTOR by amino acids and resistance exercise in skeletal muscle – PubMed, access date April 21, 2025, https://pubmed.ncbi.nlm.nih.gov/15702344/
- Mechanical Loading Modulates AMPK and mTOR Signaling in Muscle Cells – PMC, access date April 21, 2025, https://pmc.ncbi.nlm.nih.gov/articles/PMC11459513/
- Training volume increases or maintenance based on previous volume: the effects on muscular adaptations in trained males | Journal of Applied Physiology, access date April 21, 2025, https://journals.physiology.org/doi/abs/10.1152/japplphysiol.00476.2024
- The Importance of Recovery in Resistance Training Microcycle …, access date April 21, 2025, https://pmc.ncbi.nlm.nih.gov/articles/PMC11057610/
- Impact of Lower-Volume Training on Physical Fitness Adaptations in Team Sports Players: A Systematic Review and Meta-analysis – PMC, access date April 21, 2025, https://pmc.ncbi.nlm.nih.gov/articles/PMC11747014/
- (PDF) Impact of Lower-Volume Training on Physical Fitness Adaptations in Team Sports Players: A Systematic Review and Meta-analysis – ResearchGate, access date April 21, 2025, https://www.researchgate.net/publication/387728348_Impact_of_Lower-Volume_Training_on_Physical_Fitness_Adaptations_in_Team_Sports_Players_A_Systematic_Review_and_Meta-analysis
- Central and Peripheral Fatigue During Resistance Exercise – A Critical Review – PMC, access date April 21, 2025, https://pmc.ncbi.nlm.nih.gov/articles/PMC4723165/
- The 4R’s Framework of Nutritional Strategies for Post-Exercise …, access date April 21, 2025, https://pmc.ncbi.nlm.nih.gov/articles/PMC7796021/
- Sleep and Athletic Performance: Impacts on Physical Performance, Mental Performance, Injury Risk and Recovery, and Mental Health – PMC – PubMed Central, access date April 21, 2025, https://pmc.ncbi.nlm.nih.gov/articles/PMC9960533/
- Sleep and Athletic Performance – PubMed, access date April 21, 2025, https://pubmed.ncbi.nlm.nih.gov/29135639/
- Effects of exercise on sleep quality in general population: Meta-analysis and systematic review – PubMed, access date April 21, 2025, https://pubmed.ncbi.nlm.nih.gov/39556996/
- Monitoring the effects of training load changes on stress and recovery in swimmers, access date April 21, 2025, https://www.researchgate.net/publication/23134586_Monitoring_the_effects_of_training_load_changes_on_stress_and_recovery_in_swimmers
- Predicting daily recovery during long-term endurance training using machine learning analysis – PMC, access date April 21, 2025, https://pmc.ncbi.nlm.nih.gov/articles/PMC11519101/
- Physiological, Perceptual, and Performance Responses to the 2-Week Block of High- versus Low-Intensity Endurance Training, access date April 21, 2025, https://pmc.ncbi.nlm.nih.gov/articles/PMC9012527/
- The Time-Course of Acute Changes in Corticospinal Excitability …, access date April 21, 2025, https://www.frontiersin.org/journals/human-neuroscience/articles/10.3389/fnhum.2016.00607/full
- The theory of supercompensation: Strength training frequency and muscle gain, access date April 21, 2025, http://healthcorrelator.blogspot.com/2010/08/theory-of-supercompensation-strength.html
- The Science and Practice of Periodization: A Brief Review – Institute of Motion, access date April 21, 2025, http://instituteofmotion.com/wp-content/uploads/2021/01/The_Science_and_Practice_of_Periodization.pdf
- Genetic Determinants of Endurance: A Narrative Review on Elite Athlete Status and Performance – PMC – PubMed Central, access date April 21, 2025, https://pmc.ncbi.nlm.nih.gov/articles/PMC11641144/
- The Effect of Selected Polymorphisms of the ACTN3, ACE, HIF1A and PPARA Genes on the Immediate Supercompensation Training Effect of Elite Slovak Endurance Runners and Football Players, access date April 21, 2025, https://pmc.ncbi.nlm.nih.gov/articles/PMC9498790/
- Association of ACTN3 R577X Polymorphism with Elite Basketball Player Status and Training Responses – PMC, access date April 21, 2025, https://pmc.ncbi.nlm.nih.gov/articles/PMC10298064/
- Cryostimulation for Post-exercise Recovery in Athletes: A Consensus and Position Paper, access date April 21, 2025, https://www.frontiersin.org/journals/sports-and-active-living/articles/10.3389/fspor.2021.688828/full
- Types of Strength Training Periodization and Its Benefits – Vitruve, access date April 21, 2025, https://vitruve.fit/blog/types-of-strength-training-periodization-and-its-benefits/
- Periodization: Current Review and Suggested Implementation for Athletic Rehabilitation, access date April 21, 2025, https://pmc.ncbi.nlm.nih.gov/articles/PMC3438871/
- Science and Practice of Strength Training by Vladimir M. Zatsiorsky – Goodreads, access date April 21, 2025, https://www.goodreads.com/book/show/1088858.Science_and_Practice_of_Strength_Training
- V.M. Zatsiorsky – Science and Practice of Strength Training, access date April 21, 2025, https://thehubedu-production.s3.amazonaws.com/uploads/2522/e4348dcd-32e6-40aa-bf56-c6d8d4a31f63/Science_and_Practice_of_Strenght_Training_Vladimir_M._Zatsiorsky_.pdf
- (PDF) PROBLEMS OF INTEGRALITY OF SPORTS TRAINING …, access date April 21, 2025, https://www.researchgate.net/publication/389902281_PROBLEMS_OF_INTEGRALITY_OF_SPORTS_TRAINING_THEORY_BLOCK_PERIODIZATION_VERSUS_TRADITIONAL_SPORTS_TRAINING_THEORY
- Generalized training effects induced by athletic preparation A review – ResearchGate, access date April 21, 2025, https://www.researchgate.net/publication/41089383_Generalized_training_effects_induced_by_athletic_preparation_A_review
- Training periodisation – Aspetar Sports Medicine Journal, access date April 21, 2025, https://www.aspetar.com/journal/viewarticle.aspx?id=302
- Supercompensation — what’s newer literature saying? : r/StrongerByScience – Reddit, access date April 21, 2025, https://www.reddit.com/r/StrongerByScience/comments/ysm6tz/supercompensation_whats_newer_literature_saying/
- Nutritional Compounds to Improve Post-Exercise Recovery – PMC, access date April 21, 2025, https://pmc.ncbi.nlm.nih.gov/articles/PMC9736198/
- Active Recovery Induces Greater Endurance Adaptations When Performing Sprint Interval Training – PMC – PubMed Central, access date April 21, 2025, https://pmc.ncbi.nlm.nih.gov/articles/PMC6445608/
- Active recovery is better than passive recovery to optimizing post-exercise body recovery | Jurnal SPORTIF – Universitas Nusantara PGRI Kediri, access date April 21, 2025, https://ojs.unpkediri.ac.id/index.php/pjk/article/view/17685
- Active recovery is better than passive recovery to optimizing post- exercise body recovery – ResearchGate, access date April 21, 2025, https://www.researchgate.net/publication/360518452_Active_recovery_is_better_than_passive_recovery_to_optimizing_post-_exercise_body_recovery
- Active Recovery After High-Intensity Interval-Training Does Not Attenuate Training Adaptation – Frontiers, access date April 21, 2025, https://www.frontiersin.org/journals/physiology/articles/10.3389/fphys.2018.00415/full
- (PDF) Active And Passive Recovery & Acid-base Kinetics Following Multiple Bouts Of Intense Exercise – ResearchGate, access date April 21, 2025, https://www.researchgate.net/publication/7103748_Active_And_Passive_Recovery_Acid-base_Kinetics_Following_Multiple_Bouts_Of_Intense_Exercise
- Do Compression Garments Enhance the Active Recovery Process after High-Intensity Running? | Request PDF – ResearchGate, access date April 21, 2025, https://www.researchgate.net/publication/51797707_Do_Compression_Garments_Enhance_the_Active_Recovery_Process_after_High-Intensity_Running
- Compression Garments and Recovery from Exercise: A Meta-Analysis – PubMed, access date April 21, 2025, https://pubmed.ncbi.nlm.nih.gov/28434152/
- Tight Margins: Compression Garment Use during Exercise and Recovery—A Systematic Review – MDPI, access date April 21, 2025, https://www.mdpi.com/2673-7248/2/3/22
- Brain glycogen supercompensation following exhaustive exercise – PMC – PubMed Central, access date April 21, 2025, https://pmc.ncbi.nlm.nih.gov/articles/PMC3379704/
- The “supercompensation” effect of children’s lockdown during COVID-19: based on the analysis of changes in physical activity, sleep, and psychology – PubMed Central, access date April 21, 2025, https://pmc.ncbi.nlm.nih.gov/articles/PMC11154994/
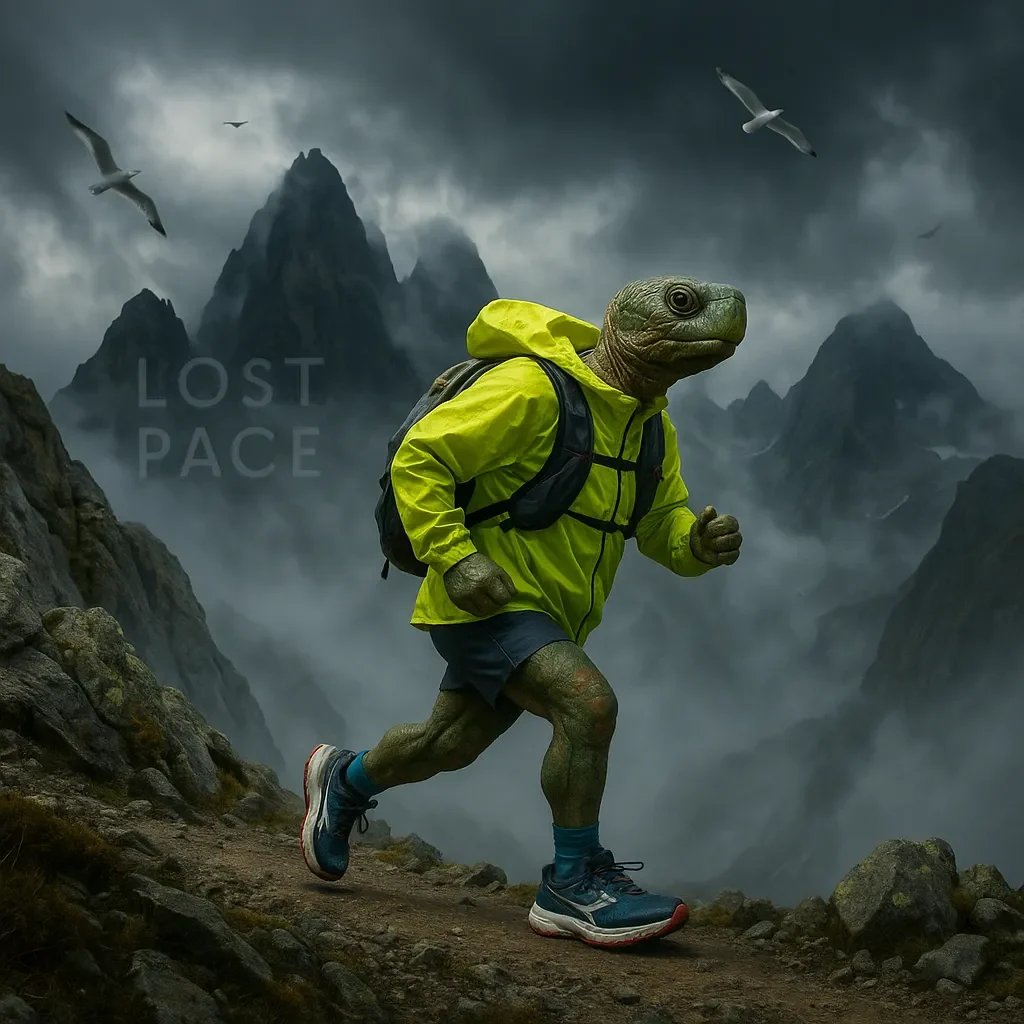
About the Author
Lost Pace is an ultramarathon runner, shoe-tester and the founder of umit.net. Based year-round in Türkiye’s rugged Kaçkar Mountains, he has logged 10,000 + km of technical trail running and completed multiple 50 K–100 K ultras.
Blending mountain grit with data, Lost analyses power (CP 300 W), HRV and nutrition to craft evidence-backed training plans. He has co-written 260 + long-form guides on footwear science, recovery and endurance nutrition, and is a regular beta-tester of AI-driven coaching tools.
When he isn’t chasing PRs or testing midsoles, you’ll find him sharing peer-reviewed research in plain English to help runners train smarter, stay healthier and finish stronger.
Ultrarunner · Data geek · Vegan athlete